[ad_1]
An improved STA of the BUNV GP permits modelling of the Gc and Gn hetero-hexameric arrangement
To determine the arrangement of Gn and Gc in the BUNV spike by cryo-EM, WT BUNV was concentrated and purified as previously described5,25 (Supplementary Fig. 1). Purified virions (in a pH 7.3/no K+ buffer) were then vitrified on cryo-EM grids. Cryo-ET tilt series were collected and 3D tomograms were reconstructed, revealing roughly spherical virions ( ~ 100 nm) in which the lipid bilayer with tripod-like spikes was clearly identifiable surrounding a core of tightly packed ribonucleoproteins (Fig. 1b), as previously described5. STA was used to align and average ~20,000 spikes, which confirmed their tripodal arrangement, resolved at ~16 Å (by gold-standard (GS)-FSC Supplementary Fig. 2a and Supplementary Table 1). In this average, both leaflets of the viral membrane (‘M’) were clearly defined at the base, with a GP spike projecting ~16 nm from the surface (Fig. 1c, d). Our STA showed distinguishable head, stalk and floor regions (Fig. 1e, f), and a previously unidentified density protruding from the floor but shielded by the tripod head (Fig. 1e white arrowheads). Fitting of the previously determined X-ray structures of the head (BUNV) and stalk (SBV) domains into our model (Fig. 1g, h) revealed a tight fit corresponding to cross-correlation values of 0.70 and 0.82 for the head and stalk domains, respectively. The fit of the head trimer allowed us to confirm the handedness of the average (Supplementary Fig. 2b, c). By exclusion, the floor domain must contain the conserved class II fusion domain of Gc and Gn, however, specific arrangements could not be obtained from this average (Fig. 1g, h and Supplementary Movie 1).
To further our understanding of the architecture of this floor domain, STA was performed focused on the floor region (Fig. 1i, j) and resulted in an average at a slightly improved resolution of ~13 Å, as determined by GS-FSC (Supplementary Fig. 2a). This average is thought to represent a hetero-hexameric assembly of a trimer of Gn and a trimer of the Gc floor region, which includes the fusion domains. In the floor domain, details were identified that were not resolved in the previously published STA5, highlighting the interconnected organisation of the fusion domains (Fig. 2a–d). Three stalk domains were also resolved, which identify the connections to three independent tripods. A post-fusion structure of the fusion domain for the related peribunyavirus LACV has been recently solved (pdb: 7A57)26. LACV Gc exhibits a class II fusion domain and shares ~49% amino acid identity and ~69% amino acid similarity with the fusion domain of BUNV Gc. We therefore modelled a LACV pre-fusion domain, based on the differences between the pre-fusion and post-fusion conformations observed for other bunyavirus class II fusion domains, which in broad terms involve the rigid-body rearrangement of domain III (Supplementary Fig. 3)9,14,27,28. In addition, there was unoccupied density remaining in the floor region that could accommodate a trimer of Gn in the centre, allowing it to contact the Gc fusion domains and the viral membrane (Fig. 2e, f).
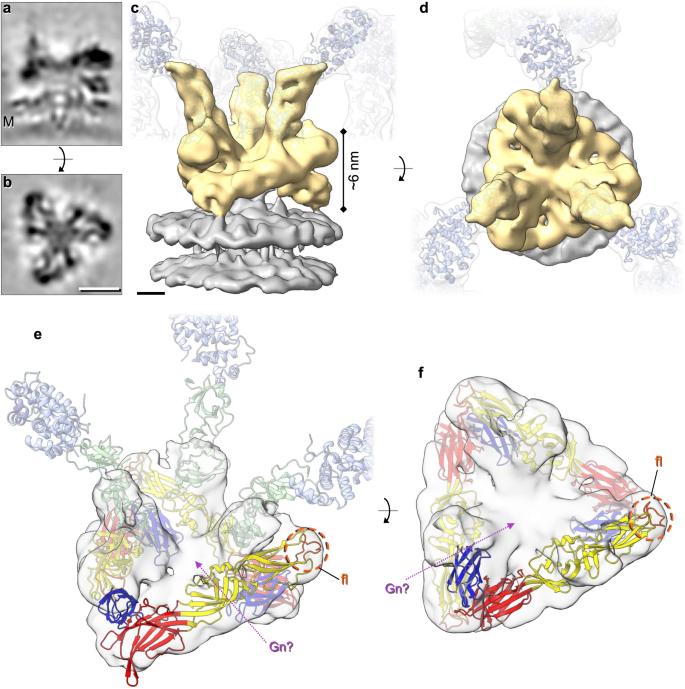
STA was performed as in Fig. 1, however aligning the floor region trimer (as opposed to the tripod). Over 16,000 subtomograms were aligned resulting in a ~ 13 Å resolution average (GS-FSC). a, b Sections through the electron density of the floor region average atop the viral membrane (M). c, d Isosurface rendering of the electron density (c side view, d top view) of the floor region (gold) atop the viral membrane (grey). Translucent tripods of the head-stalk (blue-green) regions have been added for orientation. e, f Modelling of the LACV fusion domain (residues 949–1344; domain I red, domain II yellow, domain III blue) in C3 symmetry within the BUNV floor region, revealing the location of the fusion loops (fl; orange) and remaining density that could be occupied by Gn (Gn?; pink arrow) (post-fusion structure pdb: 7A57). The location of the head (light blue) and stalk (light green) domains are indicated in (e) for orientation. Scale bars: a, b = 5 nm; c, d = 2 nm.
There are currently no Gn structures solved for any peribunyavirus, however, an AlphaFold prediction of a LACV Gn monomer was previously generated7. For Gc only partial structures are available: the BUNV head domain, SBV, LACV and OROV stalk domains, and the LACV (post-fusion) fusion domain. In order to interpret the STA map of the BUNV pre-fusion spike, we generated an AlphaFold model of the entire Gn-Gc dimeric complex (Fig. 3a, b)24,29, which reached an overall high confidence score with an average pLDDT of 85 (predicted local distance difference test, which is a per-residue estimate of its confidence; scores of >70 are expected to be modelled well24) (pLDDT scores and predicted aligned error plot in Supplementary Fig. 4a–c) with all conserved cysteines forming plausible disulphides. Fitting of the ectodomains of Gn and Gc from the AlphaFold model into our STA of the tripod (Fig. 3c, d, cross-correlation 0.72) required only minor adjustments of the angles between head/stalk and floor domains (Supplementary Fig. 4a, d; orange arrows), and it resulted in only minor clashes at protein contact sites within the floor.
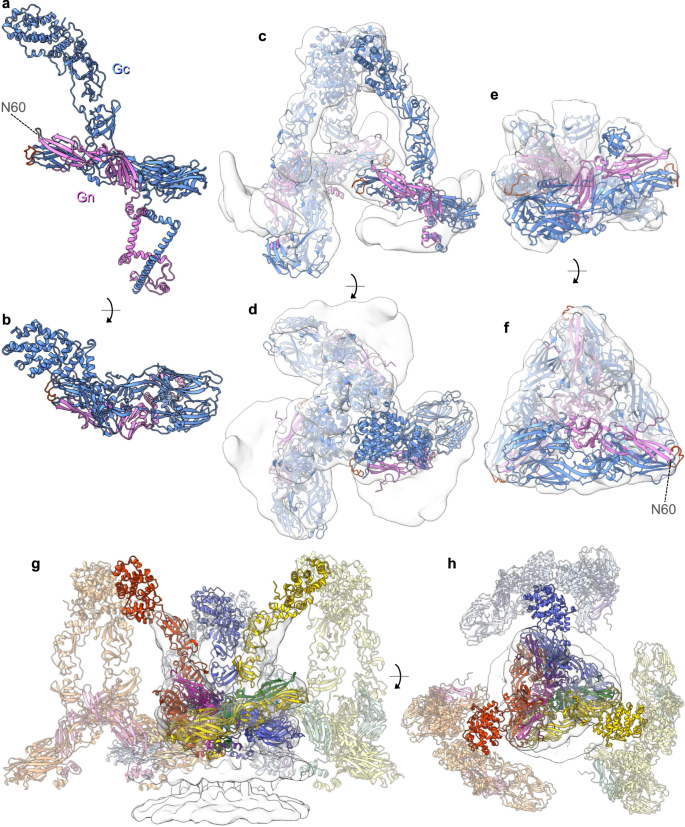
a, b AlphaFold generated an atomic model of the full-length Gn-Gc dimer. Gc in blue (fusion loop in orange), and Gn in pink with the N60 glycan on the Gn capping loop is highlighted in grey. c, d With only a minor rotation at an inter-domain region (Supplementary Fig. 4a, d), this model fitted well within the tripod STA (from Fig. 1; AlphaFold pdb found in Supplementary Data 1) in C3 symmetry (Gn-Gc ectodomains are shown; residues Gn 17-205, Gc 478-1382; no TMD). e, f The Gn ectodomain and the Gc fusion domain with the stalk subdomain II (residues 351–899) additionally fitted well within the floor region STA (from Fig. 2) in C3 symmetry. g, h A model of the BUNV GP envelope can be predicted, here showing three hetero-hexamers fitted within a floor STA. The Gn-Gc pairings are colour coded as follow; hexamer 1 purple-blue, hexamer 2 green-yellow, hexamer 3 pink-red. Supplementary Movie 2 also demonstrates this envelope model.
At the time the prediction was made, AlphaFold had not yet been trained on the recently published post-fusion structure of the orthobunyavirus LACV Gc fusion ectodomains (pdb:7A57)26, a circumstance that allowed further validation of the model. Indeed, the individual Gc fusion domains I, II, and III of the model are virtually identical to those of the LACV X-ray structure with RMSD values of 0.867-0.993 Å (pruned atom pairs; Supplementary Fig. 4e), and in the model are arranged in the canonical pre-fusion orientations relative to one another. Similarly, comparison of the AlphaFold model with the published BUNV head and SBV stalk domains revealed RMSD values of 0.419 Å and 1.087 Å respectively. The arrangement of the Gc fusion domain in the floor STA shows that a trimer fits well within this average (Fig. 3e, f; cross-correlation 0.73), and in a highly similar way to that predicted using the LACV structure with the fusion loops pointing upward underneath the tripod (Fig. 2e, f and Supplementary Movie 1).
The Gn AlphaFold model also displays the typical architecture of its orthologs from hantaviruses, phleboviruses, tospoviruses and alphaviruses, which are typically divided into four domains (domains A, B and C and the β-ribbon)7. BUNV Gn lacks the canonical domain B, but possesses domains A, C and β-ribbon observed in other bunyavirus Gn proteins7, and similarly sits parallel to domain II of the Gc fusion domain, forming numerous stabilising interactions (Fig. 4a–c). The absence of domain B makes orthobunyavirus Gn significantly shorter than its orthologues, but this appears to be compensated by the head and stalk domains on Gc, which are not found in any other bunyaviruses (Fig. 4, compare a, b, c). Our unambiguous fit of three Gn-Gc heterodimer models into the triangular floor of the spike also demonstrates that three protomers of Gn oligomerize at the centre of the floor in a similar fashion as in the tetrameric hantavirus spike9 (Fig. 4h).
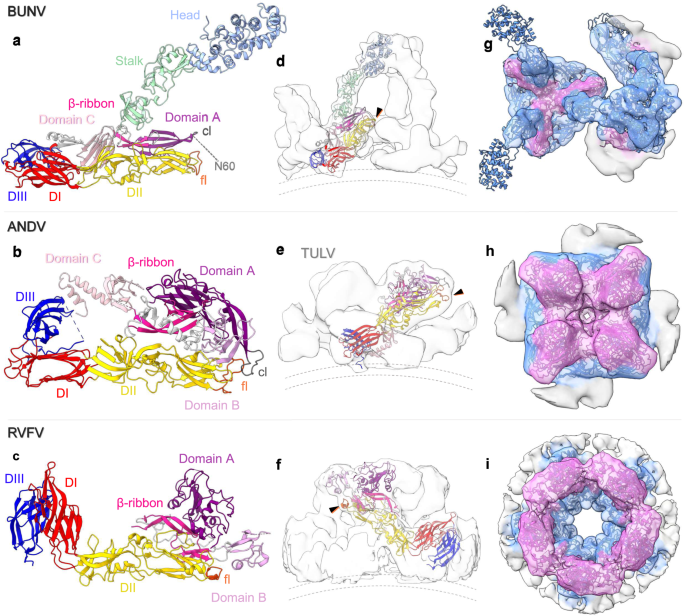
a The BUNV (orthobunyavirus) AlphaFold structure (as in Fig. 3) of Gc and Gn, as compared with the X-ray crystal structures of b the hantavirus ANDV (pdb: 6zjm) and c the phenuivirus RVFV (in the conformation as fitted within the EM average; pdb: 6f9f). The individual domain colours are the same for the three models, corresponding to the sub-domains of Gc (head (light blue), stalk (light green), domain I (DI, red), DII (yellow), DIII (dark blue) and the fusion loop (fl; orange)) and Gn (Domain A (dark-pink), Domain B (medium-pink), Domain C (light-pink), β-ribbon (magenta) and the capping loop (cl; grey)); as previously described7, 11. White corresponds to the inter-domain regions. d–f Fit of a single Gn-Gc dimer within the corresponding EM density average; side view. The black arrows indicate the position of the fusion loop and dotted lines the viral membrane. d Shows the BUNV AlphaFold fit within the floor and tripod STA (as in Fig. 3). e ANDV Gn-Gc dimer within TULV STA reconstruction (EMD-11236)9. f RVFV Gn-Gc dimer within the EM single-particle reconstruction (EMD-4201)8. g–i Top-down view of the EM density averages with fitting of the Gn-Gc multimers: g BUNV trimer (within the floor and the tripod), h ANDV tetramer, i RVFV pentamer. The density for each has been coloured by distance from residues of Gn (pink) or Gc (blue), demonstrating the relative positions of each within the complex.
Overall, the AlphaFold prediction confirms the orientation and positions of both Gn and Gc, and allowed us to model the whole orthobunyavirus GP envelope. This arrangement emphasises the role of Gn in stabilising the tripod and the fusion domains in the floor region and demonstrates how the lattice-like local arrangements are formed (Fig. 3g, h and Supplementary Movie 2).
Acidic pH and K+ alter the architecture of BUNV virions preventing virion aggregation
We are interested in the structural and functional changes of bunyavirus GPs in response to biochemical cues during entry. In our previous studies, we showed that the pH and K+ dependence of virus entry can be recapitulated in vitro (termed acid-treatment), where exposing virions to physiologically relevant endosomal [H+] and [K+], at 20 mM or higher, led to accelerated onset of infection19. Here, we aimed to untangle the effects of low pH and K+. Of note, when treating viruses in vitro at pH 6.3 prior to infection, the addition of K+ resulted in a dramatic increase in the amount of viral N protein (a known marker for viral infection) observed at 18 hpi (during the exponential growth phase), and suggested a distinct role for K+ in expediting BUNV entry19.
In order to characterise the structural effects of pH and K+ on BUNV GPs, we treated purified virions as above at both pH 7.3 and pH 6.3, and pH 6.3 with K+, prior to vitrification. 2D cryo-EM micrographs of pH 6.3/no K+ treated virions versus pH 7.3/no K+, showed that the morphology of the virions was altered and ~98% virions were forming clusters (compared to ~4% and ~13% of pH 7.3/no K+ and pH 6.3/K+ treated virions respectively, Fig. 5a, b; low magnification micrographs in Supplementary Fig. 5a, b), suggestive of direct interactions between virus particles. The electron-dense interior of individual virions can still be distinguished from one another, indicating virion-virion interactions are not fusion events. These interactions may have been driven by exposure of the fusion loops of Gc on one virion contacting neighbouring virions, leading to clustering. Intriguingly, virions at pH 6.3/K+ formed no such clusters and appeared as individual virions (Fig. 5c), suggesting that K+ prevents the direct interaction of GPs with other viruses. Taken together, these data suggest that K+ has a direct effect on virion morphology and GP architecture and function, beyond that elicited by acidic pH.
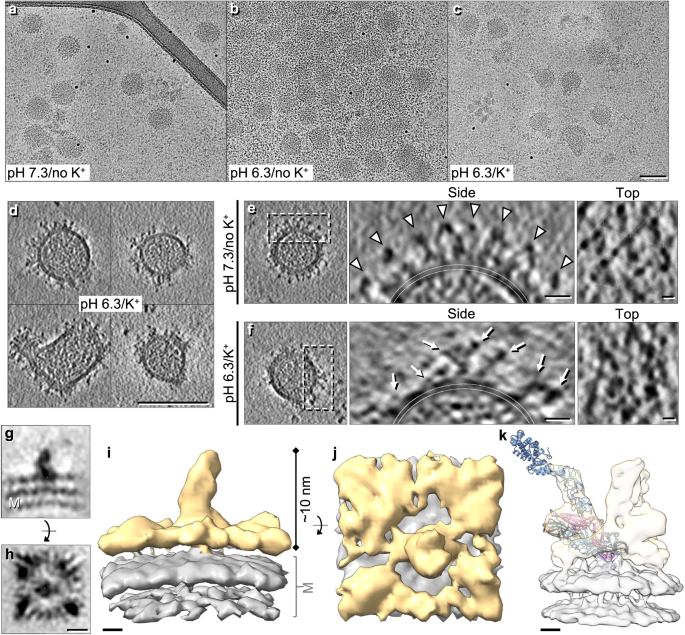
a–c Cryo-EM micrographs of BUNV virions treated under different conditions prior to plunge freezing: a pH 7.3/no K+, b pH 6.3/no K+ and c pH 6.3/K+ (n = 2, >10 micrographs per condition; low magnification images: Supplementary Fig. 5a–c) d Cryo-ET tilt series were collected of pH 6.3/K+ treated virions, then 3D tomographic reconstructions were calculated as with Fig. 1 (14 tomograms). Panels are slices through tomograms of pH 6.3/K+ treated virions which show clear disruption to the regular arrangement of GPs (representative of 89 virions, full tomogram example: Supplementary Fig. 5e). e, f An individual virion treated at e pH 7.3/no K+ (from Fig. 1) and f pH 6.3/K+ are shown, alongside a slice through the side (from the white dashed box) and top of each virion. White arrowheads in e emphasise the trimeric architecture and local lattice, and white arrows indicate spike ‘ends’ that no longer appear connected in tripods. g–j STA of ~10,000 subtomograms which were manually selected (owing to sample heterogeneity) from the surface of pH 6.3/K+ virions. g, h Slices through the centre of the spike electron density from the side (g) and top (h). The viral membrane is indicated (M). i, j Isosurface rendering of the density in g,h, with the spike (gold) on top of the viral membrane (grey). k Scaled comparison of the pH 7.3/no K+ floor region STA with the AlphaFold model fitted. Scale bars: a–d = 100 nm; e, f = 10 nm; g, h = 5 nm; i–k = 2 nm.
Cryo-ET reveals an altered GP arrangement in pH 6.3/K+ virions
We next employed cryo-ET to characterise the morphology of virions treated in conditions mimicking early endosomes (pH 6.3/K+)30. Compared to pH 7.3/no K+ virions (Fig. 1b), pH 6.3/K+ virions were noticeably more pleomorphic, with clear disruption of the regular GP arrangement (Fig. 5d, f). Areas of GP-free membrane were also evident on ~48% virions. These data suggested that pH 6.3/K+ induced disruption to the locally ordered GP arrangements and allowed GPs to move more freely, revealing spike-free membrane regions.
In our tomograms, both the tripodal structure and local trimeric lattice were easily identifiable on pH 7.3/no K+ virions (Fig. 5e). However, pH 6.3/K+ virions showed a clear disruption of this arrangement and minimal evidence of tripods (Fig. 5f and Supplementary Fig. 5g), resembling the disruption previously observed in 2D cryo-EM at pH 5.15. Of note, there were no obvious differences in the cores of the virions, suggesting that the effect(s) of pH 6.3/K+ were limited to viral GPs. Taken together, these data confirm that treatment at pH 6.3/K+ leads to drastic changes in the GP structure of BUNV.
pH 6.3/K+ results in GP hetero-hexamer uncoupling, dissociating the tripod and floor domain trimers
We subsequently used STA to determine the GP arrangement from pH 6.3/K+ virions. A dramatic change in GP architecture was observed, with the lack of clear tripods and the flexibility of the spikes evident early during processing (Fig. 5d, f, g–j). A short spike-like projection was aligned extending from the membrane ( ~ 10 nm), with a large, flat region of density above the lipid bilayer, which did not form the regular floor domain structure observed in the pH 7.3/no K+ condition. The lack of density at the top of the spike was indicative of a highly dynamic unstructured region, suggesting multiple conformations as the density cannot be well refined by STA (Fig. 5f, g). However, two features were apparent from these averages: 1) the lack of organised tripods indicates uncoupling of this region, with the spike potentially corresponding to an extended Gc fusion domain (with or without Gn) or a stalk domain from the pH 7.3/no K+ average (Supplementary Fig. 6b–d); 2) a floor region was present in this average, albeit it did not appear to form the ordered trimeric arrangement at the base of the spike comparable to the pH 7.3/no K+ (Fig. 5k). Although some of the pH 6.3/K+ densities might be visually similar to pH 7.3/no K+ floor-like triangular conformations when viewed across a Z plane, the heterogeneity is still evident and any remaining symmetric floor domains are averaged out during processing indicating they are not a highly common occurrence.
In order to obtain further indirect information about the GP arrangement on pH 6.3/K+ virions, we performed neutralisation tests with a monoclonal anti-Gc neutralising antibody (mAb-742) which recognises a conformational epitope on the head domain (near glycosylation site N624, which resides on the top of the head (Fig. 1a (asterisk) and h, N624))31,32. Western blot for BUNV-N of lysates from cells infected with viruses treated at pH 7.3/no K+, pH 6.3/no K+ or pH 6.3/K+, and incubated with mAb-742, showed that this antibody neutralises infection by all treated virions (Supplementary Fig. 7a–d). This confirms that the head-stalk domains are intact and remain attached after pH 6.3/K+ treatment, which was unclear from the STA. It is likely that the pH 6.3/K+ viruses are still neutralised owing to steric hindrance, impeding the refolding of BUNV GPs or blocking the fusion loops from physically reaching the target membrane.
We additionally confirmed effects of K+, beyond that elicited by H+, in the floor region utilising a recombinant BUNV lacking the Gc head and ~70% of the stalk domains, which are replaced with eGFP (termed GFP-BUNV; Supplementary Fig. 7e)33. We previously described that for WT BUNV pre-treatment with pH 6.3/K+ resulted in increased levels of BUNV-N at ~18 hpi compared to no treatment, suggesting that pH 6.3/K+ expedited BUNV entry. Levels of pH 6.3/K+ and pH 7.3/no K+ BUNV-N expression, however, normalise by ~24 hpi, during the post-exponential plateau phase19. Here we confirmed that the GFP-BUNV mutant virus can be triggered by pH 6.3/K+ and expedite infection similar to the pH 6.3/K+ treated WT BUNV (Supplementary Fig. 7f, g), as previously described for WT19. Therefore K+ must act on the floor region (i.e., Gn and/or the fusion domain of Gc), as this is the only common component between GPs from WT BUNV and GFP-BUNV. This aligns with our observations that pH 6.3/K+ treated virions (Fig. 5f–j) lack an ordered arrangement in the GP floor domain. Together with the previous finding that the C-terminal portion of Gc is essential for virus infection34, this indicates that the floor domain of the envelope is the main determinant for virus entry in vitro.
pH 6.3/K+ exposes the fusion loops and in the presence of a target membrane can induce fusion
As the GPs are key mediators of multiple stages of viral entry, we modified the in vitro pre-infection acid-treatment assays19 to assess the effects of K+ treatment on GP interactions with host membranes. Virions were in vitro treated at pH 6.3/K+ ( + ), pH 6.3/no K+ (-) or at pH 7.3/no K+ as a control (pH 7), and the acidic buffers were diluted out prior to binding to cells at 4 °C (to block endocytosis). Any weakly bound virions were then removed by washing, and virus binding to host cells was assessed by measuring the amount of N at 24 hpi. For WT BUNV, PBS or trypsin washes efficiently remove surface-bound virions (Supplementary Fig. 8a). In the pH 7 control and pH 6.3/no K+ treated viruses, little-to-no BUNV-N was detectable at 24 hpi when virions were washed after binding at 4 °C, indicating that virions treated at these conditions do not bind strongly to the cell surface (Supplementary Fig. 8b, c lanes 1–2, and 8d). In contrast, BUNV-N was detected at similar levels to the unwashed viruses when virions were pre-treated at pH 6.3/K+, indicating that these virions bind more strongly to host cell membranes and/or receptors, and are less readily removed by washing (compare Supplementary Fig. 8c, lane 3–4, 5, and 8d). As we showed that pH 6.3/no K+ virions form clusters (Fig. 5b), we explored if clusters remain bound to the cell surface after washing. Bound virions (at an MOI of ~10) were fixed post-washing (0 hpi), then immunofluorescently labelled for BUNV-N (Supplementary Fig. 8e). This allowed us to confirm that large virion clusters are still bound, however, are likely unable to infect cells efficiently owing to this clustering.
We hypothesised that pH 6.3/no K+ and pH 6.3/K+ elicit structural changes that expose the previously shielded Gc fusion loops which facilitate contact with target membranes upon subsequent addition to cells, hence improving cell binding. Therefore, we next investigated whether these conditions are sufficient to induce fusion. For this we tested the ability of un-treated virions to fuse with the plasma membrane under acidic conditions using acid-bypass assays35. Virions were bound to cells at 4 °C, after which pH 6.3/no K+, pH 6.3/K+ or pH 5.3/no K+ acidic buffers were added for a 2 min pulse to induce fusion at the plasma membrane and compared to an untreated control, which should not fuse. Post-bypass, pharmacological inhibitors were added to inactivate any remaining surface-bound virions (TCEP) and prevent endocytic entry (NH4Cl)19,20 (Fig. 6a). Finally, infection was assessed at 24 hpi. Under these conditions, any BUNV-N signal should represent successful fusion at the plasma membrane (Fig. 6b, lanes 1-5). Of note, BUNV-N signal was detected for the acid-bypass at both pH 6.3/no K+ (lane 3) and pH 6.3/K+ (lane 4) that was not present in the untreated control or pH 5.3/no K+ conditions, indicating that these virions can bypass endocytosis and fuse at the cell membrane. The pH 6.3/K+ BUNV-N fusion band (lane 4) was also the strongest of these conditions, suggesting that although pH 6.3/no K+ can induce fusion pH 6.3/K+ is more efficient at doing so.
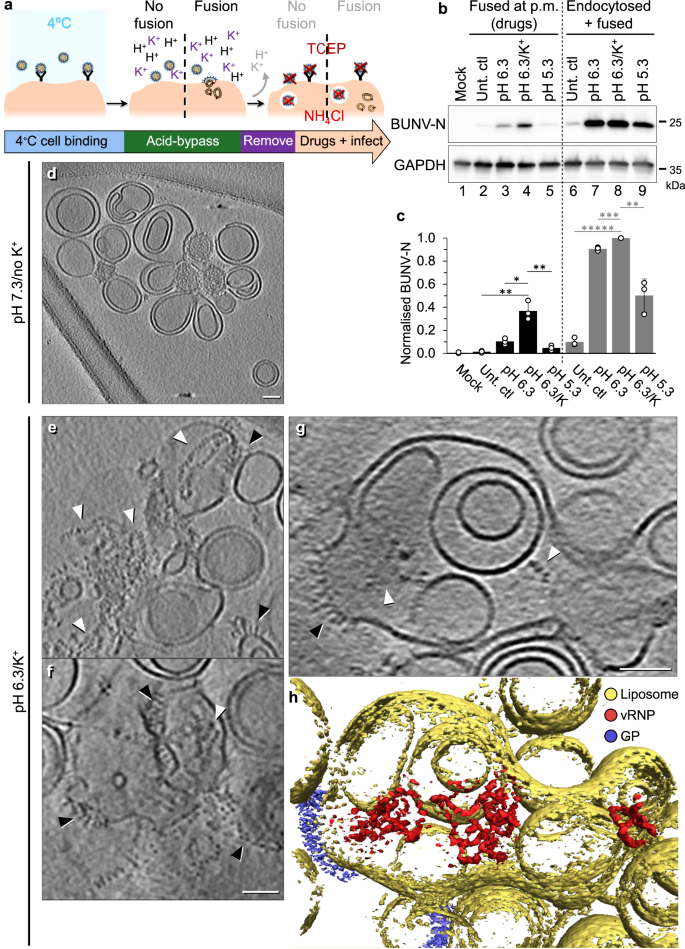
a Workflow of an acid-bypass assay. Virions are bound to A549 cells at 4 ˚C and fusion at the plasma membrane is attempted by acid-bypass at pH 6.3 (no K+), pH 6.3/K+, or pH 5.3 (no K+) for 2 mins at 37 ˚C. The addition of media for the 2 min ‘bypass’ was used for the untreated controls (‘Unt. ctl’). Bypass buffer is replaced with media with or without drugs to prevent endocytic entry (TCEP inactivates virions outside the cell; and NH4Cl inhibits endosomal acidification). Cells are lysed at 24 hpi and assessed by western blot using antibodies against BUNV-N (a marker of infection) or GAPDH (loading control). b Western blot of experiment in (a), where BUNV-N presence in lanes 2–5 (black) indicates successful fusion at the plasma membrane (p.m.) as drugs to inhibit further endocytic entry are present. In lanes 6–9 (grey) BUNV-N presence represents fusion at the plasma membrane and endocytic entry, as infection is allowed to proceed post-bypass (no drugs or harsh washing steps performed) (n = 3). c Densitometry of n = 3 western blots in (b), normalised to each loading control and then to the Unt. ctl (endocytosed+fused) (lane 6) (individual data points – white spheres). Error bars indicate mean ± standard deviation (SD), and significant difference determined using a one-way ANOVA comparing the significance of pH 6.3/K+ to each condition in the fused or endocytosed+fused (fused: Unt ctl P = 0.002, pH 6.3 P = 0.006, pH 5.3 P = 0.003. endocytosed+fused: Unt. ctl P = 1 × 10-6, pH 6.3 P = 0.0004, pH 5.3 P = 0.004). d–f Purified BUNV virions were mixed with liposomes for 2 hrs at 37 ˚C (as in Fig. 5) at d pH 7.3/no K+, or e–h pH 6.3/K+, and then samples were vitrified on cryo-EM grids (n = 3). Cryo-ET tilt series were collected and 3D tomographic reconstructions were calculated as with Fig. 1. In e–g, white arrowheads indicate vRNPs, and black arrowheads viral GPs attached to liposomes (low magnification tomograms in Supplementary Fig. 9b, c). Scale bars = 50 nm. h Tomogram segmentation of the pH 6.3/K+ tomogram in (g) was performed in Amira. Liposomes = yellow, vRNPs = red and GPs = blue (also in Supplementary Fig. 9c and Movie 4).
This was also compared to infections in the same conditions as above, but without the pharmacological inhibitors, allowing, therefore, viral entry by both fusion at the plasma membrane and by endocytosis. As a result, all corresponding BUNV-N bands are stronger (Fig. 6b, c). The limited N-band in the untreated control (lane 6) is likely owing to the short duration of virus entry during the 2 min pulse, after which non-internalised viruses are removed; and the relative higher intensity of the bands in the adjacent lanes during the western blot exposure.
To confirm that pH 6.3/K+ is inducing fusion when a target membrane is present, we incubated purified BUNV virions with artificial membranes, liposomes, at pH 7.3/no K+ or pH 6.3/K+ (37 °C, 2 hrs) to visualize fusion events by cryo-ET (similar to that previously described for influenza virus fusion30). After pH 7.3/no K+ treatment, distinct virions could be seen in close contact with liposomes (Fig. 6d and Supplementary Fig. 9a, b and Supplementary Movie 3), which are identifiable owing to their electron-dense vRNP-containing core (as in Figs. 1b and 5a). After pH 6.3/K+ treatment, fusion successfully occurred, as no distinct virions could be identified amongst the liposome clusters (Fig. 6e–h). Additionally, GP spikes could now be identified on liposomal membranes (black arrows) and vRNPs could be located within and surrounding liposomes (white arrows; free-vRNPs are likely due to liposomes breaking, either during the incubation or the freezing processes). This virus-liposome fusion is also exemplified by tomographic segmentation and 3D representation, where vRNPs can be identified within a spike-containing liposome suggesting that a virion(s) had fused with the liposome, releasing its contents within (Fig. 6h and Supplementary Movie 4). This suggests that pH 6.3/K+ and a target membrane are sufficient to induce fusion. Of note, 2D cryo-EM projections of pH 6.3/no K+ and pH 5.3/no K+ conditions also showed no evidence of whole virions reminiscent of the pH 6.3/K+ condition, suggesting fusion had occurred (Supplementary Fig. 9a), however as pH 6.3/K+ was ~3-fold stronger than without K+ at inducing fusion (Fig. 6a–c) this was the condition taken forward to cryo-ET.
Together, these data indicate that K+ and pH 6.3 act upon the BUNV GPs to promote conformational changes that increase interactions with membranes without fusion (Fig. 7b), however pH 6.3/K+ in the presence of a target membrane can induce fusion (Fig. 7a) and is the most efficient of the conditions tested.
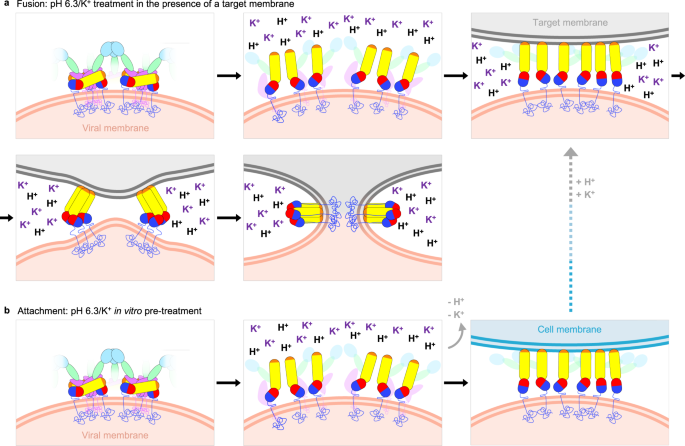
a pH 6.3/K+ in the presence of a target membrane (liposome, endosomal or plasma membrane) is sufficient to induce fusion. This is based on results from the acid-bypass assay, where the target membrane is the plasma membrane (Fig. 6a–c) and on the virus-liposome fusion observed by cryo-ET (Fig. 6e–h). The canonical model of fusion and the structural changes in class II fusion proteins are multi-step13. Instigated by exposure to a fusion trigger (e.g., pH 6.3/K+ for BUNV), the pre-fusion conformation must first dissociate in order to release the previously shielded fusion loops, generating an extended intermediate structure which can now facilitate insertion into a target membrane. The structure of the head and stalk domains is unknown at this stage, but the fact that the anti-Gc neutralising antibody mAb-742 is effective after pH 6.3/K+ suggests the structures of the individual domains remain unchanged and they remain attached to the virion. Trimerization of the fusion domains is then required for successful foldback of the fusion proteins to bring the two opposing membranes into close contact and overcome the kinetic barrier to fusion53. This then allows the two membranes to hemifuse and then fuse, involving further structural changes in the GP that lead to a post-fusion conformation, whereby the fusion loop and transmembrane domains are now inserted into the same membrane. b In the absence of a target membrane (e.g., during in vitro pH 6.3/K+ pre-treatment) the hetero-hexameric pre-fusion conformation disassembles, as seen in the cryo-ET and STA (Fig. 5f–j). This likely represents an irreversible extended intermediate conformation that is then able to insert into host membranes and bind cells more strongly (Supplementary Fig. 8c, d). Further re-introduction of H+ and K+ in the presence of a target membrane is required for fusion (as previously described19). All three conditions lead to productive fusion: target membrane, H+ and K+.
[ad_2]
Source link